Nine Challenges of Alternative Energy
Posted by Gail the Actuary on August 19, 2010 – 10:35am
Topic: Alternative energy
Tags: alternative energy, solar pv, wind [list all tags]
This is a guest post by David Fridley, known on The Oil Drum as Sparaxis. See end of post for more information.
The scramble for alternatives is on. High oil prices, growing concerns over energy security, and the threat of climate change have all stimulated investment in the development of alternatives to conventional oil. In this post (which is an excerpted chapter from The Post Carbon Reader: Managing the 21st Century’s Sustainability Crises edited by Richard Heinberg and Daniel Lerch), I give an overview of some of the issues I see, including nine challenges of alternative energy.
“Alternative energy” generally falls into two categories:
* Substitutes for existing petroleum liquids (ethanol, biodiesel, biobutanol, dimethyl ether, coal-to-liquids, tar sands, oil shale), both from biomass and fossil feedstocks.
* Alternatives for the generation of electric power, including power-storage technologies (wind, solar photovoltaics, solar thermal, tidal, biomass, fuel cells, batteries).
The technology pathways to these alternatives vary widely, from distillation and gasification to bioreactors of algae and high-tech manufacturing of photonabsorbing silicon panels. Many are considered “green” or “clean,” although some, such as coal-to-liquids and tar sands, are “dirtier” than the petroleum they are replacing. Others, such as biofuels, have concomitant environmental impacts that offset potential carbon savings.
Unlike conventional fossil fuels, where nature provided energy over millions of years to convert biomass into energy-dense solids, liquids, and gases—requiring only extraction and transportation technology for us to mobilize them—alternative energy depends heavily on specially engineered equipment and infrastructure for capture or conversion, essentially making it a high-tech manufacturing process. However, the full supply chain for alternative energy, from raw materials to manufacturing, is still very dependent on fossil-fuel energy for mining, transport, and materials production. Alternative energy faces the challenge of how to supplant a fossil-fuel-based supply chain with one driven by alternative energy forms themselves in order to break their reliance on a fossil-fuel foundation.
The public discussion about alternative energy is often reduced to an assessment of its monetary costs versus those of traditional fossil fuels, often in comparison to their carbon footprints. This kind of reductionism to a simple monetary metric obscures the complex issues surrounding the potential viability, scalability, feasibility, and suitability of pursuing specific alternative technology paths. Although money is necessary to develop alternative energy, money is simply a token for mobilizing a range of resources used to produce energy. At the level of physical requirements, assessing the potential for alternative energy development becomes much more complex since it involves issues of end-use energy requirements, resource-use trade-offs (including water and land), and material scarcity.
Similarly, it is often assumed that alternative energy will seamlessly substitute for the oil, gas, or coal it is designed to supplant—but this is rarely the case. Integration of alternatives into our current energy system will require enormous investment in both new equipment and new infrastructure—along with the resource consumption required for their manufacture—at a time when capital to make such investments has become harder to secure. This raises the question of the suitability of moving toward an alternative energy future with an assumption that the structure of our current large-scale, centralized energy system should be maintained. Since alternative energy resources vary greatly by location, it may be necessary to consider different forms of energy for different localities.
It is not possible to single out one metric by which to assess the promise of a particular alternative energy form. The issue is complex and multifaceted, and its discussion is complicated by political biases, ignorance of basic science, and a lack of appreciation of the magnitude of the problem. Many factors come into play, of which nine are discussed here.
1. Scalability and Timing
For the promise of an alternative energy source to be achieved, it must be supplied in the time frame needed, in the volume needed, and at a reasonable cost. Many alternatives have been successfully demonstrated at the small scale (algae-based diesel, cellulosic ethanol, biobutanol, thin-film solar) but demonstration scale does not provide an indication of the potential for large-scale production. Similarly, because alternative energy relies on engineering and construction of equipment and manufacturing processes for its production, output grows in a stepwise function only as new capacity comes online, which in turn is reliant on timely procurement of the input energy and other required input materials. This difference between “production” of alternative energy and “extraction” of fossil fuels can result in marked constraints on the ability to increase the production of an alternative energy source as it is needed.
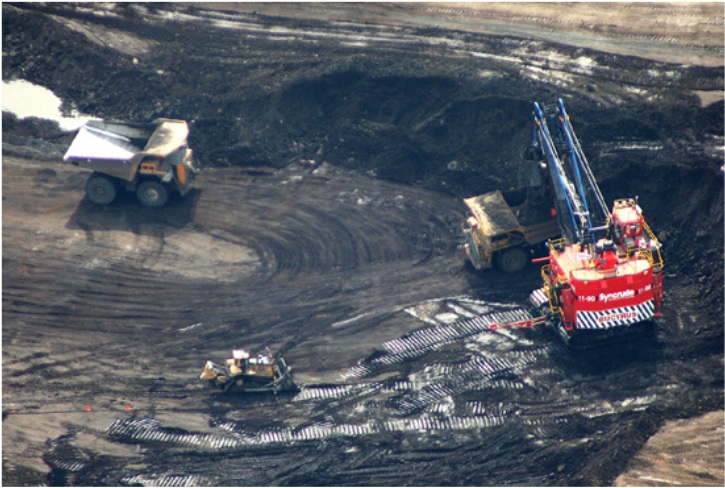
Tar sands mining in Alberta, Canada.
For example, the tar sands of Canada (although often excluded as an “alternative” energy, tar sands are subject to the same constraints because the production of oil from the tar sands deposits is essentially a mining and manufacturing operation) have already achieved a fully commercial scale of production, and because of the immense reserves indicated in Alberta, tar sands are looked to be a backstop to declining conventional crude oil production. In 2008, production of oil from the tar sands reached 1.2 million barrels per day (bpd), less than 2 percent of global production of conventional crude oil. By 2020, the Canadian Association of Petroleum Producers projects that production will increase by 2.1 million bpd to a total of 3.3 million bpd.1 But the International Energy Agency (IEA) estimates that the global decline rate from conventional-oil fields is 6.4 percent, or about 4.8 million bpd per year.2 Thus by 2020, the new oil coming from tar-sands production will not even make up half of what is being lost from ongoing depletion of existing conventional-oil fields. Even with a “crash” production program, it is estimated that tar-sands production in 2020 could not exceed 4.0 million bpd, an increase still less than the annual rate of conventional crude oil depletion.3
Scale also matters in comparing projected production of an alternative energy form against expected demand growth. In 2007, the U.S. Energy Policy Act established a target for the production of ethanol in 2022 at 36 billion gallons, of which 15 billion gallons were to be sourced from corn and the remainder from cellulosic sources. In terms of gasoline equivalency, this target is equal to 890,000 bpd of additional supply. In 2008, however, the U.S. Department of Energy, in its Annual Energy Outlook, forecast demand for gasoline would grow by 930,000 bpd by 2022,4 more than offsetting projected supply growth from ethanol and leaving gross oil dependency unchanged.
This lack of the kind of scalability needed given the magnitude and time frame of conventional-oil depletion and in the face of continued demand growth is found as well in other biofuels, coal-to-liquids, and alternative liquids for transportation. Also of concern is the difficulty of scaling up alternative energy quickly enough to meet greenhouse gas emissions targets.
2. Commercialization
Closely related to the issue of scalability and timing is commercialization, or the question of how far away a proposed alternative energy source stands from being fully commercialized. Often, newspaper reports of a scientific laboratory breakthrough are accompanied by suggestions that such a breakthrough represents a possible “solution” to our energy challenges.
In reality, the average time frame between laboratory demonstration of feasibility and full large-scale commercialization is twenty to twenty-five years. Processes need to be perfected and optimized, patents developed, demonstration tests performed, pilot plants built and evaluated, environmental impacts assessed, and engineering, design, siting, financing, economic, and other studies undertaken. In other words, technologies that are proved feasible on the benchtop today will likely have little impact until the 2030s. This reality is reflected in the key message of the now-famous Hirsch Report, which noted that to properly mitigate the economic impacts of peak oil, we would have needed to start fundamentally redesigning our national energy infrastructure twenty years in advance of the peak.5
3. Substitutability
Ideally, an alternative energy form would integrate directly into the current energy system as a “drop-in” substitute for an existing form without requiring further infrastructure changes. This is rarely the case, and the lack of substitutability is particularly pronounced in the case of the electrification of transportation, such as with electric vehicles. Although it is possible to generate the electricity needed for electrified transportation from wind or solar power, the prerequisites to achieving this are extensive. Electric-car development would require extensive infrastructure changes, including:
* Retooling of factories to produce the vehicles
* Development of a large-scale battery industry
* Development of recharging facilities
* Deployment of instruments for the maintenance and repair of such vehicles
* A spare-parts industry
* “Smart-grid” monitoring and control software and equipment
* Even more generation and transmission facilities to supply the additional electricity demand
The development of wind and solar-power electricity also requires additional infrastructure; wind and solar electricity must be generated where the best resources exist, which is often far from population centers. Thus, extensive investment in transmission infrastructure to bring it to consumption centers is required. Today, ethanol can be blended with gasoline and used directly, but its propensity to absorb water and its high oxygen content make it unsuitable for transport in existing pipeline systems,6 and an alternative pipeline system to enable its widespread use would be materially and financially intensive. While alternative energy forms may provide the same energy services as another form, they rarely substitute directly, and these additional material costs need to be considered.
4. Material Input Requirements
Unlike what is generally assumed, the input to an alternative energy process is not money per se: It is resources and energy, and the type and volume of the resources and energy needed may in turn limit the scalability and affect the cost and feasibility of an alternative. This is particularly notable in processes that rely on advanced technologies manufactured with rare-earth elements. Fuel cells, for example, require platinum, palladium, and rare-earth elements. Solar-photovoltaic technology requires gallium, and in some forms, indium. Advanced batteries rely on lithium. Even technology designed to save energy, such as light-emitting diode (LED) or organic LED (OLED) lighting, requires rare earths, indium, and gallium. Expressing the costs of alternative energy only in monetary terms obscures potential limits arising from the requirements for resources and energy inputs.
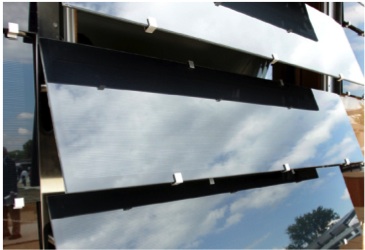
Window louvers with integrated thin-film copper indium gallium selenide
Because alternative energy today constitutes only a small fraction of total energy production, the volume of resources and energy demanded for its production has so far been easily accommodated. This will not necessarily be the case with large-scale expansion. For example, thin-film solar has been promoted as a much lower-cost, more flexible, and more widely applicable solar-conversion technology compared to traditional silicon panels. Thin-film solar currently uses indium because of its versatile properties, but indium is also widely used as a component of flat-screen monitors. Reserves of indium are limited, and a 2007 study found that at current rates of consumption, known reserves of indium would last just thirteen years.7
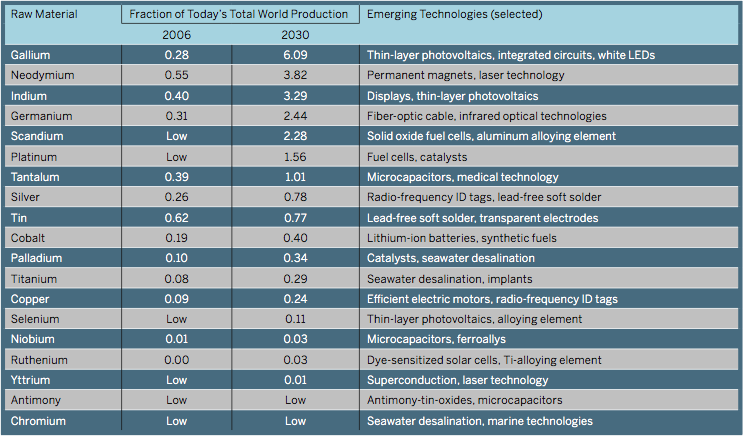
Table 18.1. Global Demand on Raw Materials from Emerging Technologies
Source: Gerhard Angerer et al., “Raw Materials for Emerging Technologies,” (Karlsruhe: Fraunhofer Institute for Systems and Innovation Research ISI; Berlin: Institute for Futures Studies and Technology Assessment IZT, February 2, 2009).
Can greatly increased demand for these resources be accommodated? As shown in table 18.1, successful deployment to 2030 of a range of new energy technologies (and some non-energy advanced technologies) would substantially raise demand for a range of metals beyond the level of world production today. In the case of gallium, demand from emerging technologies would substantially raise demand for a range of metals beyond the level of world production today. In the case of gallium, demand from emerging technologies would be expected to reach six times today’s total global production by 2030; for indium, more than three times today’s production—compared to just fractional increases in the demand for ruthenium and selenium.
Although alternative metals and materials exist for certain technologies (albeit often with performance trade-offs), embarking on a particular technology deployment path without consideration of long-term availability of material inputs can substantially raise risks. These risks are not limited to physical availability and price; they include potential supply disruptions as a consequence of the uneven geographical distribution of production and reserves. Currently, China is the dominant world source (over 95 percent) of the rare-earth element neodymium, a key input in the production of permanent magnets used in hybrid-vehicle motors and windmill turbines. In 2009, the Chinese government announced restrictions on the export of rare earths, ostensibly to encourage investment within China of industries using the metals. Whether for the rare earths themselves or for final products made from them, import dependency in the face of such a high concentration of production would do little to alleviate energy security concerns now seen in terms of import dependency on the Middle East for oil.
Alternative energy production is reliant not only on a range of resource inputs, but also on fossil fuels for the mining of raw materials, transport, manufacturing, construction, maintenance, and decommissioning. Currently, no alternative energy exists without fossil-fuel inputs, and no alternative energy process can reproduce itself—that is, manufacture the equipment needed for its own production—without the use of fossil fuels. In this regard, alternative energy serves as a supplement to the fossil-fuel base, and its input requirements may constrain its development in cases of either material or energy scarcity.
5. Intermittency
Modern societies expect that electrons will flow when a switch is flipped, that gas will flow when a knob is turned, and that liquids will flow when the pump handle is squeezed. This system of continuous supply is possible because of our exploitation of large stores of fossil fuels, which are the result of millions of years of intermittent sunlight concentrated into a continuously extractable source of energy. Alternative energies such as solar and wind power, in contrast, produce only intermittently as the wind blows or the sun shines, and even biomass-based fuels depend on seasonal harvests of crops. Integration of these energy forms into our current system creates challenges of balancing availability and demand, and it remains doubtful that these intermittent energy forms can provide a majority of our future energy needs in the same way that we expect energy to be available today.
One indication of intermittency challenges in electric power generation is the capacity factor, or the average percentage of time in a year that a power plant is producing at full rated capacity. As shown in table 18.2, photovoltaic systems produce at full capacity only 12 to 19 percent of the time over the course of a year, compared to an average of 30 percent for wind systems. In contrast, a coal-thermal plant will typically run at full capacity 70 to 90 percent of the time, while nuclear power operates at over a 90 percent capacity factor in the United States.
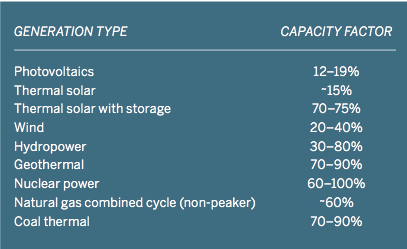
Table 18.2. Common Capacity Factors for Power Generation
Sources: Renewable Energy Research Laboratory, University of Massachusetts at Amherst, Wind Power: Capacity Factor, Intermittency; National Renewable Energy Laboratory, Assessment of Parabolic Trough and Power Tower Solar Technology Cost and Performance Forecasts, NREL/SR-550-34440 (Golden, CO: NREL, 2003).
Our current electricity system is dominated by large baseload coal- and nuclear-power generation. The integration of intermittent energy forms such as solar and wind is increasingly seen as a matter of expanding transmission capacity and grid interconnections to extend the area over which these variations are felt, as well as implementing more complex operations controls. This approach in effect relies on strengthening and expanding the large centralized energy production and distribution model that has characterized the fossil-fuel era, but may not necessarily be suitable for a future of renewable energy generation.
The key to evening out the impact of intermittency is storage; that is, the development of technologies and approaches that can store energy generated during periods of good wind and sun for use at other times. Many approaches have been proposed and tested, including compressed-air storage, batteries, and the use of molten salts in solar-thermal plants. The major drawbacks of all these approaches include the losses involved in energy storage and release, and the limited energy density that these storage technologies can achieve.
6. Energy Density
Energy density refers to the amount of energy that is contained in a unit of an energy form. It can be expressed in the amount of energy per unit of mass (weight) or in the amount of energy per unit of volume. In everyday life, it is common to consider energy density when considering food choices. Food labeling in the United States requires that both numbers needed for calculating energy density be provided: the number of food calories per serving and the weight or volume of the serving (expressed in grams or liters, respectively). Potatoes, for example, have an energy density of 200 food calories per 100 grams, or, expressed in units common in energy discussions, 8.4 megajoules8 (MJ) per kilogram (about 2.2 pounds). Cheese is more energy dense than potatoes, containing about 13 MJ per kilogram.
Energy density has also influenced our choice of fuels. Aside from alleviating a growing wood shortage, the conversion to the use of coal in the seventeenth and eighteenth centuries was welcomed because coal provided twice as much energy as wood for the same weight of material. Similarly, the shift from coal- to petroleum-powered ships in the early twentieth century was driven by the fact that petroleum possesses nearly twice the energy density of coal, allowing ships to go farther without having to stop for refueling. Even when used in a motor vehicle’s inefficient internal combustion engine, a kilogram of highly energy-dense gasoline—about 6 cups—allows us to move 3,000 pounds of metal about 11 miles.
The consequence of low energy density is that larger amounts of material or resources are needed to provide the same amount of energy as a denser material or fuel. Many alternative energies and storage technologies are characterized by low energy densities, and their deployment will result in higher levels of resource consumption. As shown in figure 18.1, the main alternatives under development to supplant gasoline use in cars are dramatically lower in energy density than gasoline itself. Lithium-ion batteries—the focus of current research for electric vehicles—contain only 0.5 MJ per kilogram of battery compared to 46 MJ per kilogram for gasoline.
Advances in battery technology are being announced regularly, but they all come up against the theoretical limit of battery density of only 3 MJ per kilogram. Low energy density will present a significant challenge to the electrification of the car fleet and will raise challenges of adequate material supply: Today, the advanced Tesla Roadster has a lithium-ion battery pack weighing 900 pounds, which delivers just 190 MJ of energy. In contrast, a 10-gallon tank of gasoline weighs 62 pounds and delivers 1,200 MJ of energy. To provide the equivalent energy to a typical gasoline car, an electric-car battery pack would need to consume resources weighing 5,700 pounds, nearly the weight of the last Hummer model.

Figure 18.1. Comparison of energy densities.
Source: Kurt Zenz House and Alex Johnson, “The Limits of Energy Storage Technology,” Bulletin of the Atomic Scientists Web edition, January 20, 2009, http://www.thebulletin.org/web-edition/columnists/kurt-zenz-house/the-limits-of-energy-storage-technology.
The more dense an energy form is, the less land is needed for its deployment. Because many alternative energies are far less energy dense than fossil fuels, large-scale deployment will incur considerable land costs. For example, a single 1,000-megawatt coal-fired power plant requires 1 to 4 square kilometers (km2) of land, not counting the land required to mine and transport the coal. In contrast, 20–50 km2, or the size of a small city, would be required to generate the equivalent amount of energy from a photovoltaic array or from a solar-thermal system. For wind, 50–150 km2 would be needed; for biomass, 4,000–6,000 km2 of land would be needed. The sprawling city of Los Angeles, in comparison, covers 1,200 km2. The land-use issue is thus a problem not only of biofuels production; siting of alternative energy projects will likely be a constant challenge because of the inherent high land footprint.
7. Water
Water ranks with energy as a potential source of conflict among peoples and nations, but a number of alternative energy sources, primarily biomass-based energy, are large water consumers critically dependent on a dependable water supply. As seen in figure 18.2, the “full-cycle” water requirement (including water for growing and processing biofuels) for key ethanol and biodiesel feedstocks is in some cases hundreds or even many thousand times higher than for the refining of gasoline. In well-watered regions with regular and adequate rainfall, much of this water can be provided through rain; in a region such as California, where no rain falls during the summer growing season because of its Mediterranean climate, irrigation is an absolute necessity for growing commercial biomass feedstocks. However, all of California’s water resources have already been allocated, so existing uses for other crops would have to be reallocated to support biomass farming—raising the issue of “food versus fuel” from yet a different angle. The water problems, however, promise only to intensify with global warming as California’s winter snowpack fades and runoff to support summer agriculture declines.
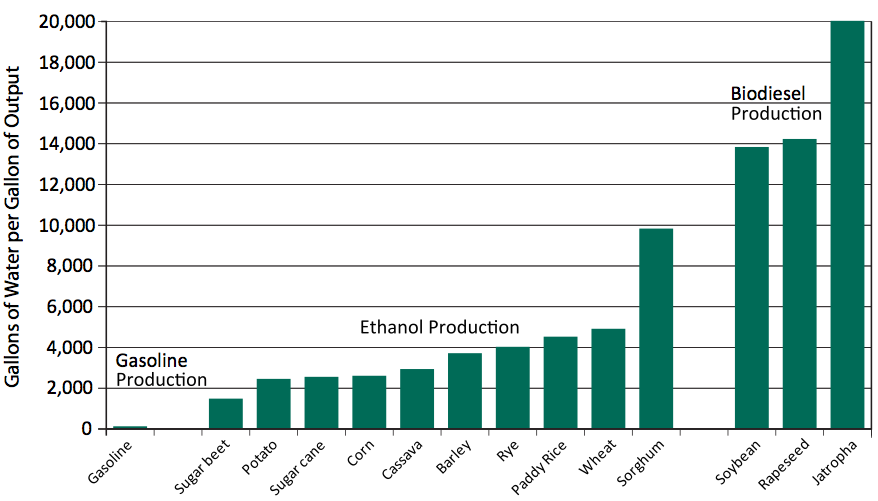
Figure 18.2. Full-cycle water requirements for biofuel production.
Source: Winnie Gerbens-Leenes et al., “The Water Footprint of Bioenergy,” Proceedings of the National Academy of Sciences 106, no. 25 (June 23, 2009), 10219–10223.
Considering just the processing stage, biomass and unconventional fossil-fuel energy also often require much greater water usage than the 2.5 gallons of water required per gallon of gasoline produced. Coal-to-liquids production consumes 8 to 11 gallons of water per gallon of output, corn ethanol requires 4 to 6 gallons, and cellulosic ethanol needs 11 gallons. In the United States, Montana has looked into becoming a leader in coal-to-liquids production, yet Montana’s dry climate suggests that water could be a limiting factor.
8. The Law of Receding Horizons9
An often-cited metric of the viability of alternatives is the expected break-even cost of the alternative with oil, or the price that crude oil would have to be to make the alternative cost competitive. Underlying this calculation, however, is an assumption that the input costs to alternative energy production would remain static as oil prices rise, thereby providing the economic incentive to development. This assumption, however, has not always proved to be the case, particularly for those alternatives for which energy itself is a major input. Because of price linkages in the energy (and now energy and biomass) markets, rising oil prices tend to push up the price of natural gas as well as coal; for processes that are heavily dependent on these fuels, higher oil prices also bring higher production costs.
A good example of this phenomenon is the assessment of the economics of production from oil shale (kerogen-rich marlstone), found in vast quantities in Colorado, Utah, and Wyoming. In the early 1970s, shale oil was expected to flood the market if the price of crude oil were to rise above $2 per barrel. When world oil prices had shot up to $35 per barrel by 1979, oil-shale production still required federal government assistance, and when oil prices fell in the mid-1980s, development and production were abandoned. Fast-forward to 2008 when oil prices moved above $100 per barrel—oil shale was then expected to be economic at $80 to $90 per barrel, and the U.S. government again provided incentives to explore production in the area. This ratcheting up of oil-shale economics with the price of oil reflects in part the high energy-input requirement to the production process.
Similarly, the corn ethanol industry has recently been subject to the same dynamic step-up in costs as the price of oil has risen. Two major input costs to the industry are the processing fuel (usually natural gas) and the corn feedstock itself. Rising oil prices after 2004 pulled natural-gas prices up as well, increasing the processing energy costs for ethanol. At the same time, higher fuel prices made cultivating corn more expensive; this, together with the additional demand for corn created by the growing ethanol industry, helped push corn prices up even further. So, although the record-high oil prices of 2008 increased demand for ethanol, some ethanol producers were operating with minimal or no profit because they had to pay more for both their processing fuel and their corn feedstock.
Ultimately, the “law of receding horizons” is a phenomenon reflective of the general orientation toward financial and economic accounting to gauge project viability and prospects. Physical accounting—that is, analyzing the material and energy inputs to a process—would help in better understanding the degree to which an alternative energy production process is vulnerable to the rise in energy costs.
9. Energy Return on Investment10
The complexity of our economy and society is a function of the amount of net energy we have available. “Net energy” is, simply, the amount of energy remaining after we consume energy to produce energy. Consuming energy to produce energy is unavoidable, but only that which is not consumed to produce energy is available to sustain our industrial, transport, residential, commercial, agricultural, and military activities. The ratio of the amount of energy we put into energy production and the amount of energy we produce is called “energy return on investment” (EROI).
This concept differs from “conversion efficiency,” which compares the amount of energy provided as a feedstock to a conversion process (such as an electric power plant or petroleum refinery) with the amount remaining after conversion. Physics dictates that this figure is always less than 100 percent. In contrast, EROI can be very high (e.g., 100:1, or 100 units of energy produced for every 1 unit used to produce it—an “energy source”) or low (0.8:1, or only 0.8 unit of energy produced for every 1 unit used in production—an “energy sink”). Society requires energy sources, not energy sinks, and the magnitude of EROI for an energy source is a key indicator of its contribution to maintenance of social and economic complexity.
Net-energy availability has varied tremendously over time and in different societies. In the last advanced societies that relied only on solar power (sun, water power, biomass, and the animals that depended on biomass), in the seventeenth and early eighteenth centuries, the amount of net energy available was low and dependent largely on the food surpluses provided by farmers. At that time, only 10 to 15 percent of the population was not involved in energy production. As extraction of coal, oil, and natural gas increased in the nineteenth and twentieth centuries, society was increasingly able to substitute the energy from fossil fuels for manual or animal labor, thereby freeing an even larger proportion of society from direct involvement in energy production. In 1870, 70 percent of the U.S. population were farmers; today the figure is less than 2 percent, and every aspect of agricultural production now relies heavily on petroleum or natural gas. The same is true in other energy sectors: Currently, less than 0.5 percent of the U.S. labor force (about 710,000 people) is directly involved in coal mining, oil and gas extraction, petroleum refining, pipeline transport, and power generation, transmission, and distribution.
The challenge of a transition to alternative energy, then, is whether such energy surpluses can be sustained, and thus whether the type of social and economic specialization we enjoy today can be maintained. Indeed, one study estimates that the minimum EROI for the maintenance of industrial society is 5:1, suggesting that no more than 20 percent of social and economic resources can be dedicated to the production of energy without undermining the structure of industrial society.11
In general, most alternative energy sources have low EROI values (see figure 18.3). Because of their high energy-input requirements, biofuels produce very little or no energy surplus.12 Similarly, tar sands provide less than 3 units of energy for each unit consumed. In contrast, wind energy shows a high return on energy investment, but it is subject to the problems of intermittency and siting issues.
>
Figure 18.3. Estimated EROI of selected alternatives.
Note: EROI measurements are not standardized; the shading indicates ranges from various studies. Abbreviations: PV = photovoltaic; CTL = coal-to-liquids; Vestas = Vestas Wind Systems.
Sources: P. J. Meier and G. L. Kulcinski, Life-Cycle Energy Requirements and Greenhouse Gas Emissions for Building-Integrated Photovoltaics, Fusion Technology Institute (2002); National Renewable Energy Laboratory, What Is the Energy Payback for PV? DOE/GO-102004-1847 (2004); Vasilis Fthenakis and Erik Alsema, “Photovoltaics Energy Payback Times, Greenhouse Gas Emissions and External Costs,” Progress in Photovoltaics 14, no. 3 (May 2006), 275–280; Luc Gagnon, “Civilisation and Energy Payback,” Energy Policy 36, no. 9 (September 2008), 3317–3322; Cutler Cleveland, “Net Energy from the Extraction of Oil and Gas in the United States, 1954–1997,” Energy 30 (2005), 769–782; Charles A. S. Hall et al., “Peak Oil, EROI, Investments and the Economy in an Uncertain Future,” in Biofuels, Solar and Wind as Renewable Energy Systems, David Pimentel, ed. (Springer Science, 2008); Vestas Wind Systems, Life Cycle Assessment of Offshore and Onshore Sited Wind Power Plants Based on Vestas V90-3.0 MW Turbines (Denmark, 2005); Alexander E. Farrell et al., “Ethanol Can Contribute to Energy and Environmental Goals,” Science 311 (January 27, 2006).
A high EROI is not sufficient to ensure that the structure of modern society and economies can be maintained, but it is a prerequisite. Unfortunately, EROI is not well understood or routinely used in energy analyses by government or industry, despite the insights it can provide. Because of the enormous investment in resources and energy that any alternative energy pathway will require, it is important that we look beyond simple financial payback, particularly in a future of rising energy prices, declining fossil-fuel resources, and increasing danger of climate catastrophe.
How Will Society Evolve in a Post-Carbon World?
Alternative energy forms are crucial for a global transition away from fossil fuels, despite the myriad challenges of their development, scaling, and integration. In face of the peaking of global oil production—to be followed by peaks in natural gas and coal extraction—and of the need to reverse trajectory in carbon emissions, alternative energy sources will need to form the backbone of a future energy system.
That system, however, will not be a facsimile of the system we have today based on continuous uninterrupted supply growing to meet whatever demand is placed on it. As we move away from the energy bounty provided by fossil fuels, we will become increasingly reliant on tapping the current flow of energy from the sun (wind, solar) and on new energy manufacturing processes that will require ever larger consumption of resources (biofuels, other manufactured liquids, batteries). What kind of society we can build on this foundation is unclear, but it will most likely require us to pay more attention to controls on energy demand to accommodate the limitations of our future energy supply. Moreover, the modern focus on centralized production and distribution may be hard to maintain, since local conditions will become increasingly important in determining the feasibility of alternative energy production.
Notes:
1Canadian Association of Petroleum Producers, Crude Oil: Forecast, Markets & Pipeline Expansions, June 2009, http://www.capp.ca/.
2International Energy Association, World Energy Outlook 2008, http://www.worldenergyoutlook.org/.
3Bengt Söderberg et al., “A Crash Program Scenario for the Canadian Oil Sands Industry,” Energy Policy 35, no. 3 (March 2007), 1931–1947.
4U.S. Energy Information Administration, Annual Energy Outlook 2008 (First Release), DOE/EIA-0383 (2008), January 2008. Note that subsequent revisions of Annual Energy Outlook 2008 changed the cited figures for gasoline demand.
5Robert L. Hirsch, Roger Bezdek, and Robert Wendling, Peaking of World Oil Production: Impacts, Mitigation, & Risk Management, U.S. Department of Energy report, February 2005,http://www.netl.doe.gov/publications/others/pdf/oil_peaking_netl.pdf.
6John Whims, “Pipeline Considerations for Ethanol,” Agricultural Marketing Resource Center, http://www.agmrc.org/media/cms/ksupipelineethl_8BA5CDF1FD179.pdf.
7David Cohen, “Earth’s Natural Wealth: An Audit,” New Scientist 23 (May 2007), 34–41.
8A megajoule equals 239 food calories; a typical adult male requires 10 MJ of food energy per day.
9As coined by user HeIsSoFly, comment on “Drumbeat,” The Oil Drum, March 7, 2007, http://www.theoildrum.com/node/2344.
10For a more in-depth discussion of energy return on investment, see Richard Heinberg, Searching for a Miracle: “Net Energy” Limits & the Fate of Industrial Society (San Francisco: Post Carbon Institute/International Forum on Globalization, 2009). Note that EROI is sometimes also referred to as “energy returned on energy invested” (EROEI).
11Charles A. S. Hall, Robert Powers, and William Schoenberg, “Peak Oil, EROI, Investments and the Economy in an Uncertain Future,” in Biofuels, Solar and Wind as Renewable Energy Systems: Benefits and Risks, David Pimentel, ed. (New York: Springer, 2008), 109–132.
12The often-cited 8:1 return on Brazilian ethanol and the high return estimated for cellulosic ethanol are not energy calculations; in these studies, the energy provided from biomass combustion is ignored. See, for example, Suani Teixeira Coelho et al., “Brazilian Sugarcane Ethanol: Lessons Learned,” Energy for Sustainable Development 10, no. 2 (June 2006), 26–39.
David Fridley has been a staff scientist at the Energy Analysis Program at the Lawrence Berkeley National Laboratory in California since 1995. He has nearly thirty years of experience working and living in China in the energy sector and is a fluent Mandarin speaker. He spent twelve years working in the petroleum industry both as a consultant on downstream oil markets and as business development manager for Caltex China. He has written and spoken extensively on the energy and ecological limits of biofuels. Fridley is a Fellow of Post Carbon Institute.
This publication is an excerpted chapter from The Post Carbon Reader: Managing the 21st Century’s Sustainability Crises, Richard Heinberg and Daniel Lerch, eds. (Healdsburg, CA: Watershed Media, 2010). For other book excerpts, permission to reprint, and purchasing visit http://www.postcarbonreader.com.
Original full article is avilable here:
http://www.theoildrum.com/node/6854